Water-holding capacity of fresh meat (ability to retain inherent water) is an important property of fresh meat as it affects both the yield and the quality of the end product. This characteristic can be described in several ways, but in fresh products that have not been extensively processed, it is often described as drip loss or purge. The mechanism by which drip or purge is lost from meat is influenced by both the pH of the tissue and by the amount of space in the muscle cell and particularly the myofibril that exists for water to reside. Numerous factors can affect both the rate and the amount of drip or purge that is obtained from the product. These factors can include how the product is handled and processed (number of cuts made and size of resulting meat pieces, orientation of the cuts with respect to the axis of the muscle cell, rate of temperature decline after harvest, temperature during storage and even the rate of freezing and temperature of frozen storage). Also of extreme importance is the metabolic state of the live animal at the time of harvest. This can be influenced by the genetic make-up of the animal and by the way the animal was handled. Ultimately, characteristics of the muscle in the live animal can have a strong influence on the amount of moisture that is lost from the resulting meat products. In summary, the entire system of live animal production and handling through initial chilling and finally storage and handling of the meat all play significant roles in influencing the amount of moisture that is lost from the product.
Introduction
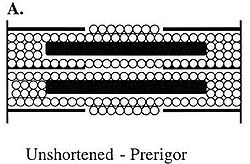
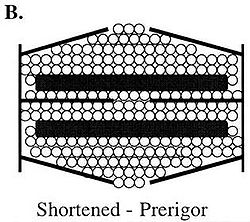
Figure B demonstrates water residing in shortened or contracting sarcomeres in pre-rigor or living muscle. Because no permanent cross-bridges have formed, the sarcomeres can expand laterally; therefore, water can remain in this structure even though the sarcomere has shortened.
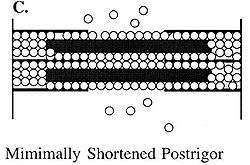
Figure C depicts the reduction in space available for water in the myofibril that occurs in postrigor muscle. Because numerous permanent rigor bonds have formed between the thick and thin filaments, the lateral space within the sarcomeres and thus within the myofibril has decreased.
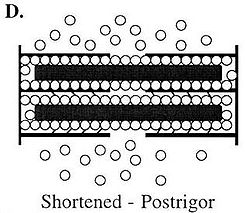
Muscle contains approximately 75% water. The other main components include protein (approximately 20%), lipids or fat (approximately 5%), carbohydrates (approximately 1%) and vitamins and minerals (often analyzed as ash, approximately 1%). The majority of water in muscle is held within the structure of the muscle itself, either within the myofibrils, between the myofibrils themselves and between the myofibrils and the cell membrane (sarcolemma), between muscle cells and between muscle bundles (groups of muscle cells). Once muscle is harvested the amount of water in meat can change depending on numerous factors related to the tissue itself and how the product is handled.
Water-holding capacity of meat is defined as the ability of the postmortem muscle (meat) to retain water even though external pressures (e.g. gravity, heating) are applied to it. The characteristic of water-holding capacity is not trivial. One of the most prevalent pork quality issues is unacceptably high moisture loss (often described as purge or drip loss) in fresh and minimally processed products. Unacceptably high moisture loss from fresh product as purge or drip has been estimated to occur in as much as 50% of the pork produced (Kauffman et al., 1992). Excess purge results in economic losses in numerous ways including reduction in salable product weight and the loss of export customers who demand high quality product with a minimum amount of purge. In addition, valuable water-soluble proteins and vitamins are lost along with moisture. Water-holding capacity of meat can also influence processing characteristics. Meat with low water water-holding capacity often tends to produce inferior processed products.
Early postmortem biochemical and biophysical processes contribute to the development of water-holding capacity. This review will focus on the physical location of water in skeletal muscle with an emphasis on possible routes of moisture escape within the structure of muscle. In addition, the factors that influence waterholding capacity of fresh meat will be reviewed.
Water in muscle
Water in muscle cells
Water is a dipolar molecule and as such is attracted to charged species like proteins. In fact, some of the water in muscle cells is very closely bound to protein. By definition, bound water is water that exists in the vicinity of non-aqueous constituents (like proteins) and has reduced mobility, i.e does not easily move to other compartments. This water is very resistant to freezing and to being driven off by conventional heating (Fennema, 1985). True bound water is a very small fraction of the total water in muscle cells; depending on the measurement system used, approximately 0.5g of water per gram of protein is estimated to be tightly bound to proteins. Since the total concentration of protein in muscle is approximately 200mg/g, this bound water only makes up less than a tenth of the total water in muscle. The amount of bound water changes very little if at all in post-rigor muscle.
Another fraction of water that can be found in muscles and in meat is termed entrapped (also referred to as immobilized) water (Fennema, 1985). The water molecules in this fraction may be held either by steric (space) effects and/or by attraction to the bound water. This water is held within the structure of the muscle but is not bound per se to protein. In early postmortem tissue, this water does not flow freely from the tissue, yet it can be removed by drying, and can be easily converted to ice during freezing. Entrapped or immobilized water is most affected by the rigor process and the conversion of muscle to meat.Upon alteration of muscle cell structure and lowering of the pH this water can also eventually escape as purge.
Free water is water whose flow from the tissue is unimpeded. Weak surface forces mainly hold this fraction of water in meat. Free water is not readily seen in pre-rigor meat, but can develop as conditions change that allow the entrapped water to move from the structures where it is found.
The majority of the water that is affected by the process of converting muscle to meat is the entrapped (immobilized) water. Maintaining as much of this water as possible in meat is the goal of many processors. Some of the factors that can influence the retention of entrapped water include manipulation of the net charge of myofibrillar proteins and the structure of the muscle cell and its components (myofibrils, cytoskeletal linkages and membrane permeability) as well as the amount of extracellular space within the muscle itself.
Composition of moisture that is lost
The aqueous solution that is lost from postmortem muscle (purge or drip) contains significant amount of protein, on average approximately 112mg of protein per milliliter of fluid. Most of the proteins found in drip are water-soluble, sarcoplasmic proteins (Savage et al., 1990). The light red color of drip is due to the fact that it contains the sarcoplasmic protein myoglobin; the pigment found in meat. Typically very little hemoglobin (pigment in blood) from blood is found in drip (Saveage et. al, 1990). In addition to myoglobin, glycolytic enzymes and other sacoplasmic proteins, amino acids and water-soluble vitamins are found in the purge.
Structure of skeletal muscle
Skeletal muscle has a very complex organization, in part to allow it to efficiently transmit force originating in the myofibrils (muscle cell organelles responsible for contraction) to the entire muscle and ultimately to the limb or structure that is moved. A relatively thick sheath of connective tissue called the epimysium encloses the entire muscle. In most muscles the epimysium is continuous with tendons that link muscles to bones. The muscle is subdivided into bundles or groupings of muscle cells. These bundles (also known as fasciculi) are surrounded by another sheath of connective tissue known as the perimysium. A thin layer of connective tissue, the endomysium, surrounds the muscle cells themselves. The endomysium lies above the muscle cell membrane (sarcolemma) and consists of a basement membrane that is associated with an outer layer (reticular layer) that is surrounded by a layer of fine collagen fibrils imbedded in a matrix.
When muscle cells are viewed under a microscope, very regular transverse striations are seen. These striations are caused by specialized contractile organelles, the myofibrils, found in muscle. The striations arise from alternating protein dense A-bands and less dense I-bands within the myofibril. Bisecting the I-bands are dark lines known as Z-lines. The area between two Z-lines is a sarcomere. The less dense I-band is made up primarily of thin filaments while the A-band is made up of thick filaments and some overlapping thin filaments. The backbone of the thin filaments is made up primarily of the protein actin. The largest component of the thick filament is the protein myosin. Myosin consists of a tail or rod region that forms the backbone of the thick filament. Myosin also contains a globular head region that extends from the thick filament and interacts with actin in the thin filament. The complex formed by the interaction of myosin and actin is often referred to as actomyosin. In electron micrograph images of contracted muscle or of post-rigor muscle the actomyosin looks very much like cross-bridges between the thick and thin filaments, indeed, it is often referred to as such. In post-mortem muscle these bonds become irreversible and are also known as known as rigor bonds. The globular head of myosin also has enzymatic activity, it can hydrolyze ATP and liberate energy. In living muscle during contraction, the ATPase activity of myosin provides energy for myosin bound to actin to swivel and ultimately pull the thin filaments toward the center of the sarcomere. This shortens the myofibril, the muscle cell and eventually the muscle to produce contraction. The myosin and actin can disassociate when a new molecule of ATP is bound to the myosin head. In post-rigor muscle, the supply of ATP is depleted, so these actomyosin bonds become permanent.
Within the structure of the muscle, there are several “compartments” from which drip could originate. These could include the space within the myofibril, the intracellular space outside the myofibril and the extracellular space, including the space between the muscle bundles. Loss of water from each of these compartments may involve slightly different mechanisms. In addition, loss of water from each of these compartments may occur at different times during storage. For example, it would be easy to envision that water found in the extracellular spaces could be lost more easily, with deeper compartments taking more time or force to be released.
Physical/biochemical factors in muscle that affect water-holding capacity
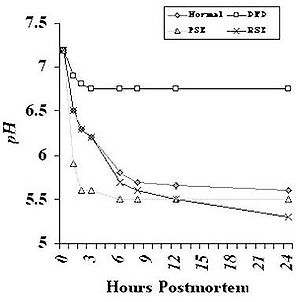
Net charge effect
During the conversion of muscle to meat, lactic acid builds up in the tissue leading to a reduction in pH of the meat. Once the pH has reached the isoelectric point (pI) of the major proteins, especially myosin (pI=5.4), the net charge of the protein is zero, meaning the numbers of positive and negative charges on the proteins are essentially equal. These positive and negative groups within the protein are attracted to each other and can result in a reduction in the amount of water that can be attracted and held by that protein. Additionally, since like charges repel, as the net charge of the proteins that make up the myofibril approaches zero (diminished net negative or positive charge) repulsion of structures in the myofibril is reduced allowing those structures to pack more closely together. The end result of this is a reduction of space within the myofibril.
Steric effects
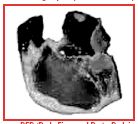
DFD (Dark, Firm, and Dry) – Dark in color, Firm in texture, and a Dry surface. Very high water-holding capacity, very little or no drip loss, very high ultimate pH. Usually caused by longterm stress which can be the result of improper live animal handling.
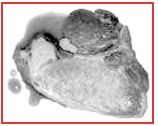
RFN (Red, Firm and Normal) – Red (normal) in color, Firm in texture, and Normal (very little) water-holding capacity, minimal drip loss Moderate rae of pH decline resulting in a normal ultimate pH ( -5.8-5.6)
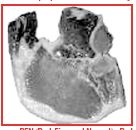
PSE (Pale, Soft, and Exudative) – Pale in color, Soft in texture, and Exudative (very wet surface) verynoticeable amount of moisture on the surface. Very poor water-holding capacity, excessive drip loss. Rapid rate of pH decline resulting in a normal or slightly low ultimate pH ( -5.6-5.5). Can be caused by live animal handling, genetics and/or inadequate chilling.
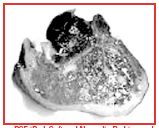
Myofibrils make up a large proportion of the muscle cell. These organelles constitute as much as 82-87% of the volume of the muscle cell. Much of the water inside living muscle cells is located within the myofibril. In fact, it is estimated that as much as 85% of the water in a muscle cell is held in the myofibrils. Much of that water is held by capillary forces arising from the arrangement of the thick and thin filaments within the myofibril. This water can be affected by changes in volume as muscle undergoes rigor. As muscle goes into rigor, cross-bridges form between the thick and thin filaments, thus reducing available space for water to reside. Additionally, during rigor development sarcomeres can shorten; this also reduces the space available for water within the myofibril. Also as mentioned previously, the net charge of the protein structures of the myofibril can also influence the amount of space available in the myofibril for water.
All of the above processes influence the amount of water in the myofibril. But, shrinkage of the myofibrillar lattice alone could not be responsible for the movement of fluid to the extracellular space and ultimately out of the muscle. The myofibrils are linked to each other and to the cell membrane via proteinacious connections. These connections, if they are maintained intact in postmortem muscle, could transfer the reduction in diameter of the myofibrils to the muscle cell (Kristensen and Purslow, 2001).
Thus, shrinkage of the myofibrils could potentially influence the volume of the muscle cell itself (Offer and Knight, 1988). In fact, reductions in the diameter of muscle cells have been noted in meat. This water that is expelled from the myofibril and ultimately the muscle cell eventually collects in the extracellular space. Several studies have shown that gaps develop between muscle cells and between muscle bundles during the post-rigor period (Offer and Cousins, 1992). These gaps between muscle bundles are the primary channels by which purge is allowed to flow from the meat; some investigators have actually termed them “drip channels”.
Factors influencing drip
Genetics and Early Postmortem Handling – Much of the potential for water-holding capacity or drip loss is truly established very early in the life of the product. In some instances, the genetics and the handling of the live animal can play a large role in influencing the future water-holding capacity of that product. However, the way the product is handled (particularly with respect to cooling) as it enters rigor also plays a critical role in influencing the amount of moisture that will be retained in the product. All of these factors (genetics, live animal handling and early postmortem temperature management) have the potential to greatly influence the rate and extent of pH decline, and thus the water-holding capacity of the meat. Therefore, it is critical that all levels of the industry understand how these factors can interact to affect water-holding capacity.
Rate of pH decline
Accelerated pH decline and low ultimate pH are related to the development of low water-holding capacity and unacceptably high purge loss. Rapid pH decline resulting in ultimate or near ultimate pH while the muscle is still warm causes the denaturation (loss of functionality) of many proteins, including those involved in binding water. The most severe purge or drip loss is often found in PSE (Pale, Soft, and Exudative) product from pigs that have inherited a mutation in the ryanodine receptor/calcium release channel (halothane gene) in the sarcoplasmic reticulum. This mutation results in impairment of the ability of this channel to control calcium release into the sarcoplasm of the muscle cell, particularly under periods of physical stress. Accelerated release of calcium causes rapid contraction and an increase in the rate of muscle metabolism and in the rate of pH decline. This particular mutation in the halothane gene can be identified in parent stock. Because a commercial test for this mutation exists, the United States industry has virtually eliminated this gene in most commercial herds.
The Halothane gene is but one example of a condition that can result in PSE. Other factors can cause PSE meat to occur. Before harvest, short-term stress in normal animals can accelerate their metabolism enough that the postmortem metabolism in the muscle is accelerated, causing a more rapid pH decline than is seen in non-stressed animals. While the condition may not be as severe as that caused by the Halothane gene, protein denaturation does occur, and drip losses can be greater than in muscle that has a normal, slower rate of pH decline. It should be noted that while the pH of these muscles falls faster than normal, the ultimate pH may not be below normal ranges.
Pre-rigor temperature
The denaturation of protein is not dependent on pH alone. The major reason rapid pH decline has such a detrimental effect on muscle proteins is because acidic pH values are attained while the muscle is still warm. It is the combination of relatively acidic conditions and near body temperatures that combine to denature protein and impair its functionality. Rapidly lowering the temperature of the carcass can mitigate some of the effects of lower pH. In addition, lowering the temperature of the meat slows metabolic processes and reduces the rate of pH decline. Therefore, chilling meat as soon as possible after exsanguination is one way to effectively alter the rate of temperature and pH decline. By slowing the rate of pH decline, the severity of the denaturation and subsequent loss of functionality of proteins is reduced and the water-holding capacity of the meat may be improved. Slowing the rate of pH decline using early and intensive chilling may prevent mild cases of PSE by avoiding the combination of both high temperature and low pH. However, in severe cases of PSE (usually caused by the presence of the Halothane gene), the rapid pH drop makes it difficult to lower the muscle temperature fast enough to prevent protein denaturation.
Ultimate pH
The pH that is attained after the muscle is in rigor has an influence on the water-holding capacity (drip loss) of meat. Meat that has a very high ultimate pH (i.e. > 6.3) tends to be dark in color and the surface of the meat appears relatively dry. This dark, firm and dry product has a very high water-holding capacity. The product is also very firm textured and it can be difficult to discern the separations between bundles of muscle fibers visually. This product is produced when the animal experienced long-term pre-harvest stress and was harvested before it could rest and replenish these stores. This long-term stress results in depletion of glycogen in the muscle. Since glycogen is the substrate for lactate production in muscle, the less glycogen that is present at harvest, the less lactate is produced after harvest, and subsequently the less the pH will decline in postmortem muscle.
On the other end of the spectrum, very low ultimate pH (5.4-5.3) can result in meat that has relatively greater drip loss than product with a normal ultimate pH (5.6-5.8) (Lonergan et al., 2001). One explanation for this low pH can be the presence of a high level of glycogen in the muscle. An estimate of the amount of glycogen can be made by measuring the amount of glycogen and its major metabolites in muscle immediately prior to or soon after slaughter. The value that is obtained is referred to as glycolytic potential (Monin and Sellier, 1985). A high glycolytic potential indicates that the muscle had a relatively large amount of glycogen at slaughter and/or had a genetic abnormality that resulted in dysfunction of an enzyme pathway involved in glucose metabolism. An example of a genetic condition that can be one cause of high glycolytic potential in pigs is the RN (Rendement Napole) gene. Pigs with this mutation tend to store more glycogen in their muscle. Pork from pigs with this gene undergoes a normal rate of pH decline after exsanguination, but because there is more glycogen, the pH continues to decline longer than normal resulting in the lower pH. The resulting meat appears normal in color or slightly lighter in color (but not to the extent seen in PSE meat), but has greater drip loss.
Processing Factors
In addition to live animal management and early postmortem handling of the meat, specific factors that may be imposed on the product after the completion of rigor can certainly impact water retention. Some of these factors include storage time, physical disruption of the product and storage conditions.
Time postmortem
In general, very little drip loss occurs in pre-rigor meat. However, at later times after slaughter, after the muscle has gone into rigor, the drip losses tend to increase (Jolley et al., 1980-81). One reason for this is that as muscle goes into rigor, the formation of rigor bonds reduces the amount of space available for water to reside in the myofibril. Also, as the muscle reaches rigor, the pH of the tissue nears the isoelectric point of many of the major proteins (especially myosin), thus influencing the amount of water that is attracted to protein structures in the myofibril. These two factors combined could greatly increase the amount of drip loss.
Another possible explanation resides in the structure of the muscle cell itself. As the pH of the muscle declines as muscle is converted to meat, the intricate latticework of the myofibril within the muscle cell shrinks, reducing the space within the myofibril where water can reside. If the proteinacious linkages between the myofibrils themselves and between the outermost myofibrils and the muscle cell membrane are intact, this shrinkage can be translated into constriction of the entire muscle cell, thus creating channels between cells and between bundles of cells that can funnel drip out of the product (Offer and Knight, 1988). These linkages between adjacent myofibrils and myofibrils and the cell membrane are made up of several proteins known as intermediate filament proteins and intermediate filament associated proteins. These intermediate filaments attach to the cell membrane via other cytoskeletal proteins. Proteins that make up, or are associated with the intermediate filaments include desmin, filamin, and synemin. Many proteins are found in the cytoskeleton at the cell membrane, some of the more notable ones are dystrophin, talin and vinculin. Degradation of some of these proteins has been shown to be related to decreased drip loss (Kristensen and Purslow, 2001; Rowe et al., 2001), especially if that degradation occurs early postmortem (Dodge et al., 2002).
Number of cuts and size of the pieces
In an intact muscle, very little drip occurs. Thus, while evaporative losses from the surface of the carcass may occur, actual drip losses from carcasses are minimal. However, once the muscles are cut the opportunity for drip to escape exists.
The size of the piece of meat can also affect the percentage of the product that is lost as drip. Smaller cuts of meat lose relatively more drip than do larger pieces of meat.(Zarate and Zaritzky, 1985). In essence, it is thought that the shorter the distance to the surface of a piece of meat, the greater the percentage of drip that is lost, even though the absolute amount of drip lost may be small compared to a larger cut of meat. This is especially true when the longest cut is across the muscle cells rather than along them, because drip tends to flow along the length of the fibers (Offer and Trinick, 1988).
Storage Conditions
Several factors during storage can also influence the water-holding capacity of meat. One of these is the storage temperature. The importance of lowering the carcass temperature as quickly as possible has been emphasized. However, it is also important to maintain the temperature of fresh meat as low as possible (without freezing) to maintain water-holding capacity. For example, increasing the storage temperature from 0 to 4°C can cause a significant increase in drip loss (Sayre et al, 1964).
Freeze/Thaw
Freezing and thawing of fresh meat can have a profound impact on the amount of moisture that is lost as drip. In fact some studies have reported that frozen and thawed pork can have almost a twofold increase in drip loss compared to the non-frozen pork (Penny, 1975). This is in part due to the physical disruption caused by ice crystals formed in the meat. Ice begins to form when meat reaches a temperature of approximately -1°C. At -5°C approximately 75% of the water in meat is ice. Maximum ice formation occurs at -20°C, at which point about 92% of the water in meat is ice. The remaining 8% is relatively resistant to freezing at temperatures even as low as -35°C (Cooke and Wien, 1971). This fraction of water is thought to be the fraction that is closely associated or bound to the proteins in the tissue. The conversion of water to ice has a profound effect on the chemical characterisitics of the meat. As ice forms, the solutes in the tissue essentially become more concentrated. At -15°C, it has been estimated that the concentration of solute in the liquid phase of the meat at that temperature is about 2M, several fold more concentrated than in fresh, non-frozen meat.
The rate at which the product is frozen can have a large impact on the amount of drip that is lost upon thawing. If the meat is frozen very quickly (flash frozen for example) the quality of the resulting thawed product may be better and less drip loss may be incurred than in product that is frozen very slowly. This is because very rapid freezing favors the formation of small ice crystals, while slow freezing favors the development of larger ice crystals. These larger ice crystals can actually cause expansion and even rupture of the cell membrane. Most of these larger ice crystals initially form outside the muscle cell as the solute concentration is less in the extracellular space and thus the freezing point is less in the extracellular space (Bevilaqua et al., 1979). Nonetheless, these crystals can be “jagged” or rough in shape and can cause significant damage to the cell membrane (Love and Haraldsson, 1961)
Conclusion
Ultimately, the entire meat production system, from the selection of genetics through storage and handling of the meat product can influence the water-holding capacity of meat. It is therefore incumbent upon each segement of the production chain to optimize their operation to reduce moisture loss from the products. This coordinated effort is needed to ensure production of an economically viable, desirable, high quality fresh meat product.
Recent Comments